High Alpha-Linolenic Acid Intake; Detrimental to DHA Status?
This article at a glance
- The conversion of alpha-linolenic acid (ALA) to longer chain and more desaturated omega-3 polyunsaturated fatty acids (omega-3 LCPUFA), in particular to DHA, is limited in humans consuming a Westernized diet.
- A recent intervention study that evaluated the effect of high ALA intake during 3 months in adults with a low baseline EPA+DHA status has observed that DHA levels in red blood cells actually decrease.
- Bioactive metabolites formed from DHA displayed variable temporal patterns of change in blood levels following ALA intake.
- This study confirms a previously documented and poorly appreciated effect of high ALA intake, namely the possibility that it compromises DHA status in people with a Westernized dietary pattern.
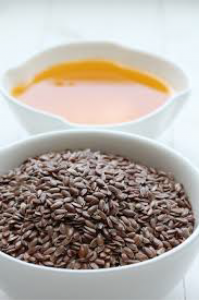
Alpha-linolenic acid (ALA), the main omega-3 polyunsaturated fatty acid found in terrestrial plants, is an important fatty acid present in the human diet. It can be converted in the body to longer chain and more highly unsaturated omega-3 polyunsaturated fatty acids, such as eicosapentaenoic acid (EPA), docosapentaenoic acid ɷ-3 (DPA ɷ-3), and docosahexaenoic acid (DHA). People display variable increases in blood and tissue EPA levels from ALA intake, but not in DHA. The conversion of ALA to DHA seems restricted in many people and is often termed “inefficient”. Although many factors, such as genetic polymorphisms, influence the expression and activities of the enzymes involved in the conversion of ALA to DHA, one reason for the poor conversion is believed to be the relatively high level of linoleic acid (LA) present in the average Westernized diet consumed today by a large proportion of people worldwide. LA competes with ALA for the delta-6 desaturase enzyme involved in the desaturation of both ALA and LA. LA is ingested in approximately 4 to 27-fold higher amounts than ALA in many countries. For as long as the dietary intake of LA is substantially higher than that of ALA, there will be a predominant conversion of LA. The same enzyme is also employed for the direct formation of DHA from DPA omega-3, and possibly in the formation of DHA from 24-carbon intermediates, representing other steps where the relative and absolute levels of LA and ALA can affect the biosynthesis of omega-3 LCPUFA.
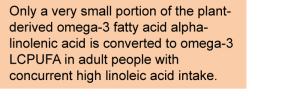
In order to satisfy the bodily needs of EPA and DHA, which vary by life stage, these fatty acids are recommended by various organizations to be obtained directly from the consumption of omega-3 LCPUFA-rich foods, such as fatty fish and seafood. In effect, this bypasses the endogenous conversion of ALA. Several studies have determined that the fractional conversion of ingested ALA to DHA in humans ranges from less than 0.1% to 5%. The rest may be used for the generation of energy and stored in adipose tissue. In normal adults consuming a western diet, it is now accepted that with no changes in the diet, improvements in DHA status cannot be achieved by supplementation with ALA. The studies that showed that only a very low portion of ingested ALA is converted to DHA, were carried out with ALA doses between 1 and 3.5 g per day. However, few studies have explicitly addressed the situation when ALA intake is much higher, i.e. higher than, or comparable to, the amount of LA ingested to achieve tissue concentrations (for example in the liver) at which ALA could effectively compete with the desaturation of LA. Under such conditions ALA could lead to higher concentrations of omega-3 LCPUFA, including DHA. A clear demonstration that ALA may, or might not, function as a precursor for DHA is important for making adequate recommendations about the intake of ALA as a suitable approach to increasing low omega-3 LCPUFA blood and tissue levels in people or for specific groups such as vegans, who completely rely on ALA conversion for the provision of omega-3 LCPUFA. In a small study, a high ALA dose (15.4 + 7.5 g) ingested for 42 days, did not increase DHA levels in plasma and platelet phospholipids in healthy male vegetarians, although the levels of EPA and DPA ɷ-3 increased. The ratio of ALA to LA intake that was achieved was 1.0:1.2.
In order to determine the effect of high ALA intake on EPA and DHA levels in humans, a recent intervention trial was carried out in adults with a relatively low omega-3 LCPUFA status. The study was performed by Greupner and colleagues from the Institute of Food Science and Human Nutrition, at the Leibniz University in Hannover, Germany. Nineteen volunteers received a daily dose of 22.3 g linseed oil, providing 14.0 ± 0.4 g ALA per day. Linseed contains ALA in the range of ~48-58% of fatty acids. The study participants (20-40 years, mean age 26.2 years) were recruited through advertisements and constituted a homogeneous population with regard to sex (all male), education level, BMI (20-27 kg/m2), a normal blood lipid profile, and having a diet that was mixed but low in meat and fish (2 or fewer portions a week) consumption. People with chronic diseases, or who had recently taken lipid-lowering drugs were excluded. The intervention period was 12 weeks, and the fatty acid composition of red blood cells, expressed as microgram (μg) fatty acid per ml of blood, was determined at baseline and at 1, 3, 6 and 12 weeks. The relative abundance of fatty acids in red blood cells (RBCs) was expressed as a percentage of total fatty acids. At baseline, EPA+DHA in RBCs was 4.63 +- 0.19% of total fatty acids, which was relatively low. Participants were asked to abstain from fatty fish and ALA-rich foodstuffs starting 4 weeks before study onset and throughout the intervention period. Three-day dietary recall interviews were held at week 0, 6 and 12, to collect data on energy and nutrient intake.
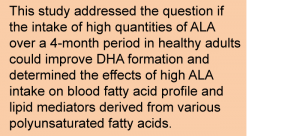
The ALA intake at baseline was 1.39 ± 1.31 g/d and increased to 13.9 and 14.0 g/d at week 6 and 12, respectively, of the intervention. The intake of LA (range 9.1 to 9.6 g/d), AA, EPA, DPA ɷ-3 and DHA remained constant during the study. Total PUFA intake increased (approximately doubled) as a result of the intake of linseed oil, whereas the intake of saturated fatty acids decreased approximately 15% during the intervention period. Total energy intake remained constant during the study. During the course of the study no statistically significant changes occurred in body weight, BMI, blood pressure and blood lipid levels (except a passing decrease in LDL level after 1 week).
ALA was readily incorporated into RBC membranes; from a baseline level of 1.44 ± 0.10 μg/ml, its level increased to 6.25 ± 0.24 μg/ml at week 6 and 5.80 ± 0.28 μg/ml at week 12. The ALA supplementation also led to parallel increases in EPA (almost doubling in level from 6.13 ± 0.51 to 11.0 ± 0.64 μg/ml at week 12) and DPA ɷ-3 (approximately 25% increase from 25.9 ± 0.82 μg/ml to 32.3 ± 1.35 μg/ml). The increased levels of ALA, EPA and DPA ɷ-3 were accompanied by a small decrease in the level of AA, that was largest after 12 weeks. Linoleic acid levels, which made up >10% of total RBC fatty acid content, did not change. Unexpectedly, the level of DHA in RBCs significantly decreased nearly 25% (from a baseline level of 41.0 ± 1.93 μg/ml to 30.4 ± 1.09 μg/ml at week 12).
While the relative sum of EPA and DHA amounts did not change significantly in response to high ALA intake, the total omega-6 PUFA decreased and the ratio of AA to EPA levels significantly decreased. The increase in both EPA and DPA ɷ-3 levels significantly contributed to an increase in the %n-3 in highly unsaturated fatty acids (HUFA), even though the DHA level had decreased markedly. In other words, a marked shift in the profile of omega-3 LCPUFA occurred in response to high ALA intake. During the 8-week follow-up period after the end of the intervention period, the levels of the various fatty acids started to return to their baseline values, although it was apparent that a much longer period would be required for full return.
The effect of the high ALA intake on the levels of ALA-, EPA-, DHA, AA- and LA-derived oxylipins (enzymatically-oxygenated derivatives), was determined in plasma by liquid chromatography-mass spectrometry (LC-MS)-based targeted metabolomics. Oxylipins formed from ALA and EPA increased, whereas those from AA decreased, reflecting the respective changes of the parent fatty acids. The concentrations of LA-derived oxylipins in plasma did not change, consistent with the lack of change of LA levels. In contrast, the changes in DHA-derived oxylipins were not consistent. The level of 22-hydroxy-DHA (22-HDHA) decreased at 12 weeks, whereas there were no changes in 17- and 20-HDHA levels during the intervention period, and the levels of 4-HDHA and 7-HDHA significantly increased.
The results of this study indicate that in male adults ingesting a Westernized diet, ALA is not a good fatty acid source to increase DHA in RBCs. High ALA intake also did not increase the sum of both EPA and DHA but did increase both EPA and the more abundant omega-3 fatty acid DPA ɷ-3. DPA ɷ-3 is not always reported in studies assessing fatty acids or by diagnostic read-outs of omega-3 LCPUFA status, such as the omega-3 index that is increasingly employed to document tissue levels of omega-3 LCPUFA. In the current group of study participants, DPA ɷ-3 levels were four times higher than EPA levels.
Whether high ALA intake actually lowers the tissue concentrations of DHA in organs other than RBCs cannot be concluded from the present study. It is plausible that DHA is displaced from RBC membranes by the augmented incorporation of ALA and by the increased formation of both EPA and DPA ɷ-3. Selective redistribution of RBC DHA to other organs causing a net decrease in RBC DHA levels is however not very likely since omega-3 LCPUFA levels in different lipid pools are in equilibrium. It is also plausible that high ALA intake could directly inhibit the desaturation of DPA omega-3 to DHA, or activated the retroconversion of DHA to DPA ɷ-3 and EPA. After ALA supplementation was stopped, the level of DPA ɷ-3, but not of EPA, in RBCs still increased for another 4 weeks, possibly pointing to continued retroconversion of DHA to DPA ɷ-3. The increased formation of several DHA-derived oxylipins may also suggest that high ALA intake selectively stimulates the further oxidative biotransformation of DHA, possibly contributing to a net decrease in RBC levels, but the mechanism whereby activation of specific oxygenation routes of DHA occurs, while other routes of oxidative metabolism are down-regulated, is a matter for future investigation.
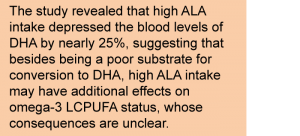
A limitation of the study was that there was no control group. Rather, the study was set up as a longitudinal repeated-measures study, where each individual served as its own control. Another limitation is the reliance on food recall interviews to determine nutrient intakes by participants, which is prone to errors but is a recognized limitation in many dietary intervention studies. On the other hand, a strength of this study is the meticulous documentation of the quantitative changes of fatty acid intake and fatty acid profiles, and a range of downstream lipid mediator products.
Linseed oil contains ~ 48-58% ALA, but also contains LA (range ~11-16%). Given the background dietary intake, LA intake was still in the range of 9.25 g/d (baseline) to 9.58 g/d at week 12. Therefore, it is possible that the relative availability of LA is still too high compared to ALA and might effectively compete with one biosynthetic conversion step between EPA and DHA. The result would be an increase in EPA and DPA ɷ-3, but no efficient formation of DHA. A similar experiment where high ALA is ingested on the background of a very low dietary intake of LA might provide an answer to the question if ALA can, under very specific circumstances, still be an efficient precursor for DHA biosynthesis. For human omnivores with mixed diets that are relatively rich in LA, a situation reflective of most people in the industrialized world, such a scenario is very difficult to achieve. In adults, there are two ways to increase blood or breastmilk DHA: consume DHA, or decrease LA. For example, increased conversion of ALA to DHA was shown in a study with women who had recently given birth, in whom a relatively high dose of 10.1 g/d ALA from chia oil replaced their usual oil intake from the sixth month of pregnancy to the sixth month of nursing. LA intake decreased from 16 to 9 g/d over the intervention period, and significantly increased levels of DHA in breast milk were observed. In contrast, perinatal supplementation of >10 gram/day of ALA on top of the current diet does not support DHA formation and levels in breast milk.
The demonstration that high ALA intake alone may furthermore depress endogenous DHA levels may stimulate some new discussion on the perceived importance of the need for ALA in our diet as an “essential” fatty acid precursor for omega-3 LCPUFA formation in adults with a Westernized lifestyle. Any biological functions of ALA itself are furthermore challenging to study in people because the conversion of ALA to the more highly desaturated and elongated fatty acids is impossible to eliminate completely. This study provides a potential alert that, in the context of concurrent high LA intake, a high ALA intake, which could result from the dietary intake of certain food products rich in ALA, might even be detrimental to omega-3 LCPUFA status as a result of an unexpected and paradoxical decrease in DHA levels. Whether this occurs in all tissues, how this occurs, and if this is in fact harmful to health will need to be addressed in new research.
Greupner T, Kutzner L, Nolte F, Strangmann A, Kors H, Han A, Schebb NH, Schuchardt JP. Effects of a 12-week high-α-linolenic acid intervention on EPA and DHA concentrations in red blood cells and plasma oxylipin pattern in subjects with a low EPA and DHA status. Food Funct. 2018;9(3):1587-1600. [PubMed]
Worth Noting
Bayrak A, Kiralan M, Ipek A, Arslan N, Cosge B, Khawar KM. Fatty acid compositions of linseed (Linum usitatissimum l.) genotypes of different origin cultivated in Turkey. Biotechnol. Biotechnol. Eq. 2010;24(2):1836-1842. [Link]
Burdge GC, Calder PC. Conversion of alpha-linolenic acid to longer-chain polyunsaturated fatty acids in human adults. Reprod. Nutr. Dev. 2005;45(5):581-597. [PubMed]
Chan JK, McDonald BE, Gerrard JM, Bruce VM, Weaver BJ, Holub BJ. Effect of dietary alpha-linolenic acid and its ratio to linoleic acid on platelet and plasma fatty acids and thrombogenesis. Lipids 1993;28(9):811-817. [PubMed]
Francois CA, Connor SL, Bolewicz LC, Connor WE. Supplementing lactating women with flaxseed oil does not increase docosahexaenoic acid in their milk. Am. J. Clin. Nutr. 2003;77(1):226-233. [PubMed]
Hu XF, Sandhu SK, Harris WS, Chan HM. Conversion ratios of n-3 fatty acids between plasma and erythrocytes: a systematic review and meta-regression. Br. J. Nutr. 2017;117(8):1162-1173. [PubMed]
Lands B, Bibus D, Stark KD. Dynamic interactions of n-3 and n-6 fatty acid nutrients. Prostaglandins Leukot. Essent. Fatty Acids 2017;Jan 25. [PubMed]
Li D, Sinclair A, Wilson A, Nakkote S, Kelly F, Abedin L, Mann N, Turner A. Effect of dietary alpha-linolenic acid on thrombotic risk factors in vegetarian men. Am. J. Clin. Nutr. 1999;69(5):872-882. [PubMed]
Park HG, Park WJ, Kothapalli KS, Brenna JT. The fatty acid desaturase 2 (FADS2) gene product catalyzes Δ4 desaturation to yield n-3 docosahexaenoic acid and n-6 docosapentaenoic acid in human cells. FASEB J. 2015;29(9):3911-3919. [PubMed]
Valenzuela R, Bascunan K, Chamorro R, Barrera C, Sandoval J, Puigrredon C, Parraguez G, Orellana P, Gonzalez V, Valenzuela A. Modification of docosahexaenoic acid composition of milk from nursing women who received alpha linolenic acid from chia oil during gestation and nursing. Nutrients 2015;7(8):6405-6424. [PubMed]